Capacitive Plasma Parameters
Chamber Pressure
The voltage necessary to initiate a discharge is roughly a function of the product of the spacing between electrodes and the pressure. The minimum voltage occurs at a product of about 1 Torr-cm. At higher pressures, the discharge voltage increases, making it difficult to start the plasma if the electrode spacing is large.
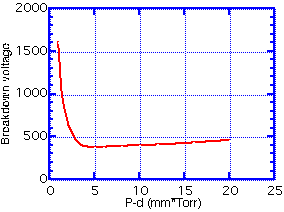
ref: Basic Data of Plasma Physics, S. Brown, American Inst. of Physics Press
At very low pressure (or more properly pressure-distance product), there are too few collisions and electrons traverse the chamber and strike the walls without ionizing. Again the voltage for initiating the discharge increases. For typical chamber geometries, it is very difficult to initiate a capacitive discharge at pressures less than 10-20 mTorr, though it is often possible to "strike" the discharge at higher pressure and then operate at only a few mTorr. This high breakdown voltage is exploited in making dark space shields, grounded plates placed within a few mm of a powered electrode to localize the plasma above the electrode.
The electron and ion diffusivity increase just like neutral diffusivities as roughly (1/P). [The diffusion of electrons and ions is, however, coupled; we'll discuss this ambipolar diffusion later.] Thus at pressures of 10's of mTorr, electrons diffuse readily and the plasma tends to spread through the reactor; at pressures of a few Torr and above plasmas are generally confined to the regions where the electric fields heat the electrons.
Recombination of electrons and ions requires a third body (e.g. another molecule) to carry away the energy of ionization -- otherwise, the electron will just fly off again. Since the likelihood of a third body being around just when needed goes up with pressure, recombination in the gas is very slow at low pressures (a few Torr and below) but can be important in high pressure plasmas (greater than about 10 Torr for typical geometries).
Time and Frequency
What frequency of excitation should one apply to the electrodes to create a plasma? What difference does it make?
DC or AC?
As we've noted, electrons and ions are lost primarily to the walls at pressures of a few Torr or less, rather than to recombination in the gas phase. For chamber sizes of a few cm (shortest length) the electrons are gone in 20-100 µsec. Thus:
f < 10 KHz =DC: plasma off between cycles
f > 100 KHz =AC: plasma on continuously
AC or RF?
Typical ion velocities are similar to typical molecular thermal velocities, on the order of 105 cm/s. For a typical sheath thicknesses of 0.5 - 2.5 mm, ion transit times are 0.5 - 2.5 µsec. Thus:
f < 1 MHz : ions cross the sheath in < 1 RF cycle, follow instantaneous sheath potential (an AC plasma)
f > 10 MHz : ions take many RF cycles to cross the sheath, see time-average potential (an RF plasma)
At low frequencies, ion energies can vary from very small values (when the sheath is small ) to energies equal to the peak RF voltage. At 13.56 MHz, the range in ion energies is much reduced, because the ions gain energy over several RF cycles.
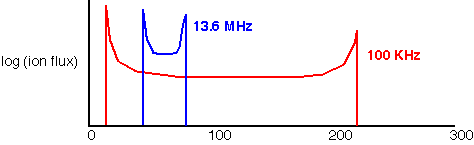
calculated for Ar plasma; reference: van Roosmalen et. al., Dry Etching for VLSI 1991 p. 30
Keeping It Going
The plasma is sustained by hot electrons which strike molecules to knock off another electron, creating an ion. At very low frequencies (< 10 KHz) the mechanism for creating these hot electrons is very similar to that operating in DC plasmas: the large sheath voltage present at the cathode accelerates the secondary electrons, which gain enough energy to ionize molecules in the plasma. This is an inefficient process: very large sheath voltages (400-700 V) are required, and much of the electron energy is dissipated in non-ionizing collisions.
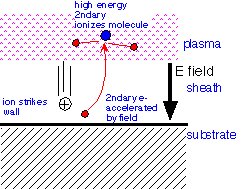
As frequency increases into the MHz range, two new mechanisms for transferring energy to electrons become important. First, the change in sheath sizes with each RF cycle requires that charge move back and forth through the plasma -- that is, that a displacement current flow must exist. This displacement current, like any other current, encounters some resistance as it flows in the plasma, and leads to a voltage and thus heat dissipation through P = V*I. Since the current is proportional to frequency, and the power is proportional to the square of the current, the amount of power dissipated scales as the square of the frequency.
Displacement current heating takes place in the bulk plasma, and is most important at high pressures and large electrode gaps.
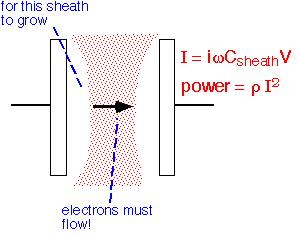
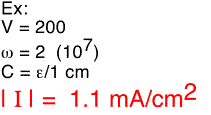
The motion of the sheath is itself significant: a 1 cm wide sheath growing and shrinking 10,000,000 times per second must be moving at about 107 cm/second! Such a velocity is quite comparable to electron thermal velocities. Electrons in the plasma can "scatter" from the sheath and gain energy. Naturally, they can also give up energy to the sheath when it is moving away from them, but the number of electrons encountering the sheath is higher when it moves into the plasma than when it moves out.
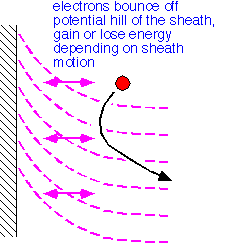
Sheath reflection is localized near the moving sheath edge, and is especially important for low pressure plasmas (10's to 100's of mTorr). It also scales as the square of the frequency.
The Mystery Number: It is very common for an excitation frequency of 13.56 MHz to be employed in plasma processing. This frequency, and some of its harmonics, are reserved for industrial as opposed to communications use by the FCC. While there is no underlying physical reason to prefer 13.56 MHz as opposed to, say, 12 MHz or 14.5 MHz, the powerful influence of the economics of scale has made power supplies and matching networks more widely available at lower cost for this standard frequency than for others nearby.
Reactor Geometry: Electrode Areas
In many practical reactors, one electrode is "grounded" -- connected to the chamber wall, which is normally connected to "true" ground for safety reasons. In this case, the area of one electrode is much larger than that of the other.
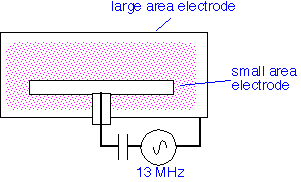
In such reactors, it is empirically found that most of the RF potential appears across the sheath near the smaller electrode. Simple theoretical arguments predict a dependence on the fourth power of the electrode area, but a quadratic dependence is more typically observed in practice.
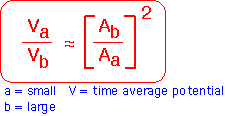
As a consequence, larger peak ion energies are observed on a substrate mounted on the smaller electrode. This configuration is sometimes referred to as a reactive ion etch configuration, and distinguished from the plasma etching configuration with either substrates mounted on the large area, or equal area electrodes.
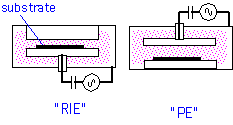
Many plasma CVD processes operate at a high enough pressure (a few Torr) that the plasma is mostly contained between the electrodes, so that they are effectively symmetric reactors, even if the wall area is actually large.
RF Power and Multiple Frequency Excitation
In a conventional reactor, we can increase the RF power to get a higher ion bombardment energy, but the plasma density will also increase. We can't adjust these two parameters independently.
An additional degree of flexibility can often be obtained by providing more than one frequency of excitation of a capacitive plasma. A typical approach is shown schematically below. Two separate power supplies are employed, each attached to one electrode. Filtering is employed to minimize the interaction between the two signals: in this case, we've shown an inductor that grounds the top electrode at 100 KHz, while appearing to be a high impedance for a 13 MHz signal. Similarly, a capacitor is used to ground the lower electrode for high frequency signals. This configuration is sometimes known as a triode reactor. Alternative configurations where both supplies are connected to the same electrode can also be employed.
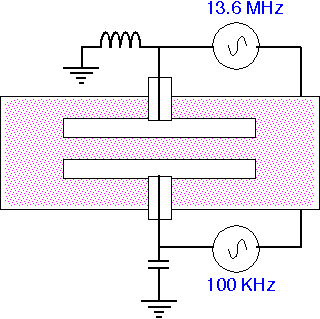
To a fair approximation, the high frequency power controls the plasma density, due to the more efficient displacement current and sheath heating mechanisms mentioned above. The low frequency excitation influences the peak ion bombardment energy, as discussed previously. Therefore, the user has some ability to separately adjust the ion bombardment energy and the plasma density, which is not very easy with a single excitation energy. Reactors of this design have found applications in both CVD and plasma etching. In particular, adjustment of the low-frequency / high-frequency power split allows control of deposited film stress, valuable for silicon oxides and nitrides (see our discussion of CVD Films for more details).
Some (now old) papers on dual frequency reactors:
"Advantages of Dual Frequency PECVD for Deposition of ILD and Passivation Films" E. van de Ven, I. Connick and A. Harrus, VMIC 1990
"A Dual Frequency Plasma Sheath Model" F. Myers and T. Cale, J. Electrochem. Soc. 139 3587 (1992)
"Prediction of Ion Energy and Angular Distribution in Single and Dual Frequency Plasmas"; F. Myers, M. Ramaswami and T. Cale
J. Electrochem. Soc. 141 p. 1313 (1994)
"Plasma impedance and microwave interferometric measurements of electron concentrations in dual-frequency powered sulfur hexafluoride plasmas"
V. Jaikprakash and B. Thompson, J. Vac. Sci. Technol. A12 p. 1403 (1994)
Return to Tutorial Table of Contents
Book version of the CVD Tutorial