The world has come a long way since I sat slicing up tiny silicon solar cells with a dicing machine at International Rectifier in 1977. Back then, solar photovoltaic cost of $1 per peak watt (that is, on a clear day with the sun shining down on the panels at noon) was the hallucinatory dream of visionaries suspected of indulging in 1960s-style drugs. Today the cost of large-scale installations, including not just the devices but also the mounting, voltage conversion, and solar tracking, is flirting that magic $1/peak watt goal. Wind energy has followed a similar path. At this cost level, renewable electricity costs about the same as energy from classic fossil fuels. Looking forward, the problem is not collecting energy without burning something, but getting that energy where and when it is needed: energy storage and transport.
Historically most energy storage has been through materials that can burn in our oxygen-containing atmosphere and release heat. The common media have been wood, coal, petroleum, and natural gas. In each case, we get the storage for free. Wood stores sunlight and does so when the sun shines. If we don't cut down the trees too soon (a big problem in many societies throughout history), we can collect the result at modest cost. Since we didn't pay for the energy, it doesn't matter that much that it is captured rather inefficiently. Coal, petroleum, and natural gas are even more extreme examples: the energy stored in these materials is the result of sunshine from hundreds of millions of years ago that we collect today. Burning wood sufficed to provide energy for most of the activities of human society since the invention of fire as a tool a couple hundred millennia ago. The more recent discovery of fossil fuels propelled us to new heights of industrialization and ambition.
Unfortunately, there's a hidden price for our free energy sources. Burning forests has catastrophic ecological and human consequences, as detailed for example in Jared Diamond's book Collapse. Burning fossil fuels is taking those hundreds of millions of years of carbon capture and undoing them in the blink of eye (geologically). If we burn all the fossil fuels we know about, we will at the least return the earth to carbon dioxide levels characteristic of the Cretaceous, when there was substantially no ice on the surface of the earth and sea levels were a couple of hundred meters higher than today. That may not be a problem for life in general, but it's a big problem for human societies mostly built around coastlines and rivers in temperate and tropical regions. Given the destructive capabilities we've equipped ourselves with, human society is unlikely to survive such a fate, and human beings themselves might go extinct, likely taking a lot of earth's living diversity with them.
To keep this sort of drama in science fiction rather than fact, we need to replace energy stored in these fossil remnants of the past with energy that we intentionally tuck away. How shall we proceed? At the most basic level, the usefulness of a way of storing energy depends on three basic characteristics: how much does it weigh? how big is it? how expensive is it? But the way those, and other, characteristics determine whether a method of storing energy is practical are very complicated and completely different for different applications.
Let's begin with weighty matters. The energy stored in a medium per unit mass is the mass storage density. We will measure it in megaJoules per kilogram, MJ/kg. A megajoule is enough energy to heat about three liters of water (a bit less than a gallon) to boiling. It is a bit more than 1/4 of another common unit of energy, the kilowatt-hour. In Figure 1 below we show the mass density for some approaches to energy storage.
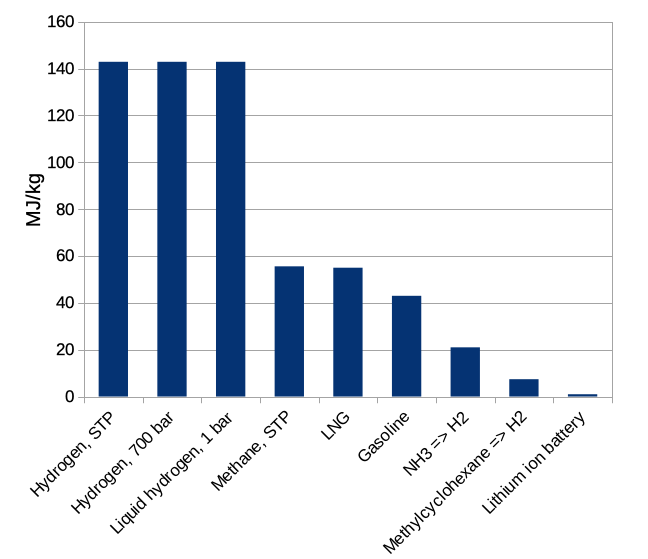
Figure 1: energy storage density per mass, MJ/kg, of some common materials and methods. STP = standard temperature and pressure (1 atmosphere and room temperature here); 700 bar = 700 atmospheres. LNG is liquified natural gas, which is mostly methane. NH3 is ammonia, considering only the hydrogen it contains as a source of energy. Similarly, methylcyclohexane is an organic compound that can be regarded as a hydrogen storage medium.
If we stopped here it would be extremely obvious how to store energy: use hydrogen! It has 3 times more energy per kilogram than gasoline, and is much better than natural gas as well. The contents of a 10-gallon gas tank (weighing about 27 kg) could be replaced with 8 kg of hydrogen, leaving more for passengers and payload. This sounds great until we recall that we should also think about how big our energy storage medium is. The energy storage density per unit volume of the same materials is shown in Figure 2.
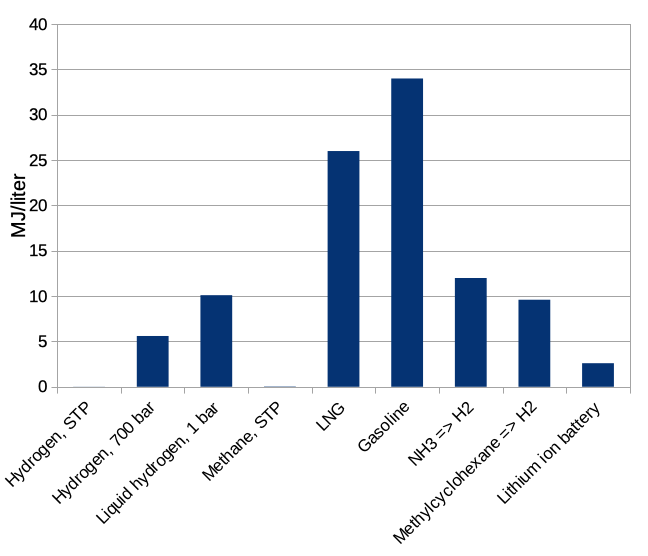
Figure 2: energy storage density per volume, MJ/liter, for the same set of materials. STP is standard temperature and pressure, here 1 atmosphere at room temperature.
We see that plain old hydrogen gas at room temperature and ordinary atmospheric pressure is — really awful. You need a huge balloon to store enough energy to accomplish anything. Compressing the hydrogen only gets you to about 7 times the volume of gasoline for the same energy stored. Chilling it down to the very low temperatures at which it is a liquid is still three times worse than a tank of gasoline. Gasoline looks wonderful, and we can see why it has been so widely employed as an energy storage medium for vehicles, where space taken matters about as much as mass.
But then when we look at the consequences for our world of using a kilogram of each fuel, we see that natural gas and gasoline are both pretty bad. If we're trying to make a society that doesn't cook our home, we need to use something else.
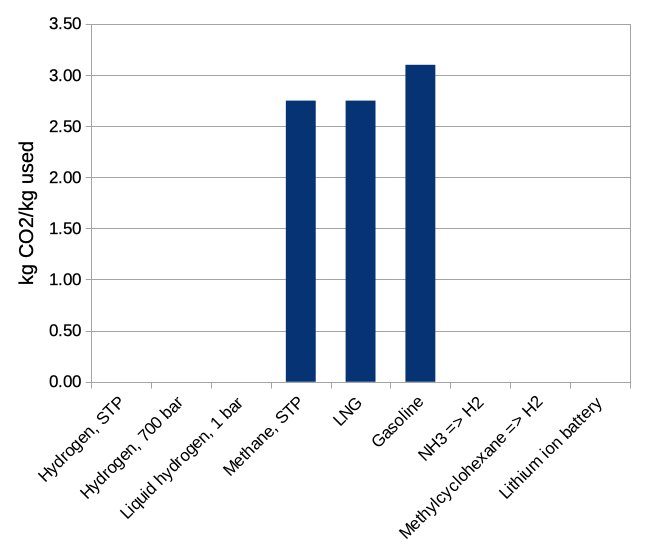
Figure 3: kilograms of carbon dioxide released by the use of 1 kilogram of each material.
You might note that our friend the lithium battery looks fairly pathetic in both the density graphs (although superior to plain old gases like hydrogen or methane). Batteries have much lower energy storage density than most chemical approaches. But they have some really nice properties for practical use. They are very efficient — you get most of the energy you put in back out. Many battery types can be recharged in place without any fancy additional chemicals or processes. The energy comes right out when you want it — just connect the battery to an electric circuit with a motor in it. (It's a bit more complex than that in a real use, like a Tesla electric vehicle, but still fundamentally straightforward.) And they don't generate carbon dioxide in use, although of course making the energy they contain might have involved carbon dioxide release. Batteries are important in a number of applications because everything needed to make them work is readily available today, but we can see that there might be applications where something else would be nicer. Batteries are fundamentally limited in energy density. It has been a struggle to get the performance of today's lithium batteries, and there is no known path for achieving mass or volume density comparable to gasoline or liquid hydrogen.
Everything shown above has been known for decades (although, as we noted, battery performance has improved somewhat during this time). The hydrogen economy was widely touted at the turn of the century as the appropriate path for our society, but twenty years later we don't see much of it around in our daily lives. What is the problem or problems, and are there solutions? To get a better understanding of what's going on, we will look at a few representative applications, to see what works, what doesn't, and how we might reach a more sustainable future.